Regeneration of joint cartilage: perspectives and future
Regenerar el cartílago articular: perspectivas y futuro
Resumen:
Los tratamientos de las lesiones del cartílago cambiaron significativamente desde la incorporación de las técnicas de biotecnología, inicialmente con el cultivo de condrocitos autólogos en un medio líquido, posteriormente con la incorporación de matrices, embebidas o no con células cultivadas. El reto continúa y esas técnicas que han mostrado unos buenos resultados clínicos deben mejorarse para establecer un protocolo que sea capaz de regenerar el tejido cartilaginoso. Desde nuestro punto de vista, los ensayos clínicos y los trabajos de investigación son primordiales, para conocer la biología articular y ser capaces de dirigir la condrogénesis.
Abstract:
The treatment of cartilage lesions changed significantly with the introduction of biotechnological developments, initially in the form of culturing autologous chondrocytes in liquid medium, and later followed by the incorporation of matrixes with or without cultured cells. The challenge continues, and these techniques, which have demonstrated good clinical outcomes, need to be improved in order to establish a protocol capable of regenerating cartilage tissue. From our point of view, clinical trials and research studies are essential in order to better understand joint biology and be able to direct chondrogenesis.
Introduction
The decisions made today, with the work done in the past, mark the future. In the case of the repair of cartilage lesions, many scientists and surgeons have worked in collaboration with patients to address the same problem and find a solution. However, a change in direction such as that we are now experiencing can occur at any time, as it has done so often in the course of history.
Many studies have been published related to cartilage repair, though not all of them are equally valuable, since few randomized and controlled clinical trials are available, and likewise there are not many meta-analyses with level of evidence 1. Most of the existing publications are cohort studies or clinical cases, characterized by subjective conclusions and with scant scientific support. Nevertheless, the proposed treatments for repairing cartilage alleviate the symptoms - fundamentally pain, improve joint function and mobility, and prevent the lesion from progressing(1), though it is curious that very diverse treatments based on very different principles reportedly afford the same outcomes.
The existing conservative management strategies include physiotherapy, pharmacological measures such as chondroprotective drugs (glycosamine sulfate, chondroitin sulfate, collagen, diacerein, etc.), and intraarticular injections of hyaluronic acid or platelet factors. Unfortunately, none of these treatments repair or regenerate the damaged cartilage. In the best of cases, they are able to alleviate pain, improve function and - possibly - slow progression of the disorder. On the other hand, the surgical techniques used to repair cartilage result in tissue substitution or reconstruction with an uncertain long-term outcome, and in most cases they likewise are unable to achieve complete regeneration of the damaged tissue, since functional hyaline cartilage is rarely generated.
One of the most interesting techniques involves the infiltration of mesenchymal stem cells (MSCs). Awad et al.(1), in a meta-analysis of 33 clinical trials involving MSC treatment to regenerate knee cartilage in 724 patients, comparing pain and joint function, found bone marrow aspirate concentrate to reduce pain and improve joint function(2,3,4).
A randomized clinical trial(5) assigned 56 patients to two groups. One of the groups received cultured MSCs with an injection of hyaluronic acid three weeks after the operation. These patients showed clinical improvement as well as improved MOCART (Magnetic Resonance Observation of Cartilage Repair) scale versus the other group that received placebo and hyaluronic acid. Furthermore, 9 of the patients administered MSCs showed a covering of cartilage tissue.
The relationship between patient age, MSC viability and the subsequent evolution of the cartilage transplant over time is another controversial issue. Buda et al.(6) considered that patient age and gender, and the size of the lesion, do not affect the outcome. Nejadnik et al.(7) designed an observational cohort study to compare cartilage repair using autologous chondrocytes (36 patients) and MSCs (36 patients). Patient age was not seen to affect the MSC group, while individuals under 45 years of age showed better outcomes in the autologous chondrocyte transplant group.
Many variables are implicated in the treatment of chondral lesions. While the lesions are similar, the treatments are very different. This circumstance makes it necessary to develop recommendations and guides based on studies with level of evidence 1 and involving long evolutive times, carefully reflecting the characteristics of the lesions and with full information on the treatments used - such data being lacking in most of the published studies. It is also necessary to assess the magnetic resonance imaging (MRI) scans with the standardized scales in order to establish a precise diagnosis with correct evaluation of evolution over time. Furthermore, the case histories of the included patients must be reflected, with their comorbidities, previous medications related to the lesion, previous surgeries, etc.
It is also necessary to know the characteristics of the chondrocytes or MSCs used, i.e., whether they come from cell cultures (cultured cells) or are obtained directly from an aspirate concentrate, as well as the total number of cells injected. If aspirates are used, the technique employed should be specified, along with the information referred to isolation and processing - including centrifugation and the surface markers used to characterize the cells. If the cells are obtained from culture (cultured cells), we need to know the times, cell passages, temperatures and culture media employed. Any publication should offer all the data referred to the technique involved, including the surgical procedure, the contents of the suspension used for cell injection, and clear specifications of any additional agent combined with the cells (dexamethasone, platelet-rich plasma [PRP]).
The trials must analyse the postoperative and rehabilitation protocols. The existing publications are characterized by a great variety of protocols and clinical assessment methods (imaging-based or histological). Likewise, the clinical and functional scales are highly varied and scantly sensitive. Imaging-based diagnosis is more consistent, and in this regard MRI is the reference technique. However, not all studies use the same sequences or employ the same assessment scales.
Considering the future, the development of new techniques for the repair and regeneration of joint cartilage must be based on prevention, biotechnology focused on specific growth factors, the development of biomarkers, gene therapy, and utilization of the mechanical regulation of cartilage - all fundamented upon in vitro and in vivo research and clinical investigations supported by correct methodology. Naturally, the surgical techniques in turn must be increasingly precise and with guarantees of being able to afford the best outcomes - though this issue is addressed by other chapters of this supplement.
Biotechnology
The repair observed after performing microfracture shows that the body is sufficiently able to achieve regeneration, even if the amount and quality of the repaired tissue are suboptimal. Techniques have been developed in recent years in this field, affording improved outcomes and better quality of the repaired tissue(8,9).
The biotechnological techniques are based on the use of cultured cells, either multipotent MSCs or chondrocytes. Different strategies have been proposed for the use of bone marrow MSCs(10); the direct intraarticular injection of autologous MSCs (cultured or otherwise) is the simplest way to release cells in the case of cartilage injury. Another option, particularly in patients with osteochondral defects, is MSC implantation in a membrane(1). However, management and manipulation with MSCs must be clarified in order to secure the best and most effective outcomes, with histological assessment of the results obtained.
In vitro and in vivo studies have shown that following the implantation of MSCs that differentiate into chondrogenic cells under the influence of growth factors, these cells tend to acquire the morphology and characteristics of hypertrophic chondrocytes concordant with those of growth cartilage or bone formed through endochondral ossification - and this may lead to the formation of calcified repair tissue(11,12). In this respect, efforts must be made to adopt factors that suppress hypertrophic differentiation and preserve the phenotype of hyaline joint cartilage.
The use of allogenic MSCs is the area that has generated most interest, and in order to assess its efficacy, Vangsness et al.(13) designed a randomized, double-blind controlled study. These authors established two groups of patients with three different doses of allogenic MSCs, and found all groups to experience improvements in pain and in the Lysholm score two years after treatment.
Growth factors
Hyaline cartilage is an avascular tissue. It is therefore difficult to expect such tissue to experience repair, and cells or signals capable of triggering the tissue regenerating mechanisms need to be supplied. The strategies developed to repair cartilage have focused on the stimulation of anabolism using anabolic growth factors or chondroinduction factors (transforming growth factor β [TGF-β], bone morphogenetic proteins [BMPs], fibroblast growth factor [FGF]). These factors can induce MSC differentiation into chondrocyte-like cells and stimulate the production of cartilage matrix. When speaking of growth factors, most publications refer to PRP, without knowing the type and number of injected factors.
Therapies based on the use of growth factors have been questioned due to the need for large doses, the short half-life of such factors within the joint, their cost, and the possibility of inducing collateral effects(14,15,16). This is because in addition to cartilage, there are other tissues within the joint that exhibit very different characteristics (synovial membrane, cancellous bone, subchondral bone, meniscus), and the application of external growth factors leads to synovial membrane hyperplasia, joint inflammation or ectopic cartilage and bone formation, with pain and loss of mobility(17). As evidenced in the case of meniscal tissue, there are even factors with opposite effects depending on whether they act upon the cells of the vascular zone or on the cells in the non-vascular zone(18).
A number of studies have shown that repeated injections of TGF-β, BMP2 or BMP9, or the adenoviral over-expression of TGF-β in the knee of mice gives rise to osteophytes(19,20,21,22,23). It is therefore not clear which are the most appropriate factors or what the indicated doses are in order to guide cell growth and differentiation.
Growth factors are normally associated with the stimulation of chondrogenesis, though under certain conditions these proteins may also exert an anti-chondrogenic effect. One known example is FGF2, which stimulates the growth and chondrogenic differentiation of MSCs derived from adipose tissue and bone marrow, but in turn counters the synergic pro-chondrogenic effect of BMP2(24,25,26).
The suppression of anti-chondrogenic factors is a way of stimulating in situ chondrogenesis on the part of joint MSCs (endogenous repair) or of releasing MSCs with a strong chondrogenic potential (cell therapy)(10). The "anti-chondrogenic" factors include proinflammatory mediators and factors that induce mature chondrocyte hypertrophy.
Few studies have focused on anti-chondrogenic regulators of help in maintaining the chondrocyte phenotype. Chondrogenesis may be inhibited physiologically at extracellular level with growth factors and inhibitors of growth factors and proinflammatory cytokines, and at transcriptional / translational level with transcriptional (co)regulators and microRNA (miRNA). In fact, many extracellular inhibitors (noggin, follistatin, gremlin and chordin) block the activity of pro-chondrogenic growth factors and act as BMP antagonists(27). Also some WNT proteins (Wnt1, Wnt4, Wnt7A, Wnt8 and Wnt9A) inhibit chondrogenic differentiation of the progenitor MSCs(28). On the other hand, GDF11, by activating NOTCH1, suppresses the expression of chondrocyte markers and the production of cartilage(29) (Figure 1).
Proinflammatory factors
Inflammatory cytokines (tumour necrosis factor-α [TNFα] or interleukin-1b [IL-1b]) exert a negative influence that affects both cartilage mechanics and the synovial fluid lubrication properties(30).
Two macromolecules - lubricin and hyaluronic acid - are primary lubricants that maintain the conditions of the synovial fluid(31,32,33). The concentrations of the inflammatory interleukin IL-1b increase in inflammatory osteoarthritis and following trauma; the over-expression of IL-1b is associated to proteoglycan loss and softening of the extracellular matrix(34,35,36). On the other hand, IL-1b also reduces the expression of lubricin(37) and increases the expression of catepsin B(36,37,38), resulting in an increase in cartilage friction(39,40).
Inflammation could be modulated by inhibiting the proinflammatory signals as a therapeutic option to reduce cartilage degeneration and create a microenvironment favourable for repair. Cartilage damage elevates the extracellular inflammatory mediators, including inflammatory chemokines and cytokines produced by the inflamed tissues themselves and released directly into the synovial fluid. These factors are essential as an initial stimulus for tissue repair, but their increase or chronic production impedes chondrogenesis and degenerates the newly formed cartilage(41). It is no surprise that some proinflammatory mediators (IL-1b, TNFα, molecules of the IL-6 and IL-8 family) are also recognized as potent anti-chondrogenic factors(42).
Transcription factors
Chondrogenesis is regulated by many transcription factors, fundamentally SOX9 and RUNX2/3, which act as basic regulators of MSCs and the development of cartilage. The inhibition of anti-chondrogenic transcription factors may be a simple strategy for stimulating the formation of cartilage through genic expression(10). Other transcription factors that also may be targeted are TWIST1, SLUG/SNAIL2, Homebox (HOX), AP-2, YAP1 (Yes Associated Protein-1) and TAZ - the latter being negative regulators of chondrogenesis(43,44).
MicroRNA
The post-transcription mechanisms act in the regulation of chondrogenesis and in the production of cartilage. The level of control is potent, since a single microRNA (miRNA) targets hundreds of mRNA. It has been reported that 169 miRNA molecules participate during chondrogenesis derived from MSCs(45), which defends the idea that many miRNA are able to exert anti-chondrogenic functions, and that such suppression is necessary in order for chondrogenesis to take place. For example, miRNA-195 exerts a focalized anti-chondrogenic effect upon FGF-18, and this promotes chondrogenesis, with an in vivo protective effect upon cartilage lesions. A number of miRNA inhibit the suppression of pro-chondrogenic transcription regulators; most of them belong to the SOX gene family. The expression of miR-145 is negatively correlated to the chondrogenic potential of the progenitors derived from induced pluripotent stem cells (iPS)(46). Likewise, miR-145, miR-30, miR-495 and miR-1247 have been characterized as anti-chondrogenic miRNA molecules that modulate SOX9(47).
Modulation of anti-chondrogenic regulators
Advances in molecular therapy and biotechnology have provided powerful tools for inhibiting the expression or function of intra- and extracellular regulators. At extracellular level, antibody block could inhibit anti-chondrogenic growth factors and cytokines, as well as the inhibiting growth factors themselves. This strategy is used in clinical practice with chemotherapy for the treatment of cancer and also in rheumatoid arthritis(48,49). At intracellular level, RNA interference (RNAi) is used to block the synthesis and function of regulatory proteins and miRNA(10).
The idea of blocking anti-chondrogenic miRNA is increasingly more widely accepted for the treatment of cartilage lesions(50). Three types of inhibitors are available for this purpose: antimiR (antagomiR), which are short oligonucleotides that sequestrate miRNA and constitute the most common option; miRNA molecular sponges that compete with mRNA for interaction with miRNA(51); and small molecule miRNA inhibitors (SMIR) such as diazobenzene, the benzothiazoles and neomycin(52), which are less popular but have the advantage of easy release and stability in a liquid medium.
Biomarkers
A future line of research and also an increasingly manifest need is the development of adequate biomarkers for joint cartilage. Such markers are to become a basic element for the diagnosis and control of the evolution of chondral lesions and involutional joint disease. A biomarker is an objective and evaluated indicator of a normal or pathological biological process, or of a pharmacological response, following a therapeutic intervention(53). There are "dry" biomarkers (imaging parameters) and "wet" biomarkers (genetic and biochemical parameters) that can be detected in blood, serum, urine or synovial fluid, and tissues.
Potential biomarkers for cartilage comprise proteins or enzymes that reflect cartilage metabolism. Work has been ongoing for some time on biomarkers capable of allowing the early identification and assessment of the grade and evolution of osteoarthrosis. The white book of the OARSI (Osteoarthritis Research International Society) was developed as a result of a call from the United States Food and Drug Administration (FDA) for the definition of biomarkers related to osteoarthrosis(54,55).
Over 50% of the osteoarthrotic patients in clinical trials show no morphological or clinical changes during two years(56); this makes it necessary to identify those individuals that will progress and quantify - or at least objectively assess - the progression of the disease. Biomarkers will serve to determine the efficacy of a treatment and identify those patients requiring treatment(57) (Figure 2).
Matrix metalloproteases (MMPs) comprise a large family of proteases with a broad range of substrates including extracellular components, cytokines, receptors and cell motility factors(58,59). In this context, MMP-1 or stromalysin-1 is produced by chondrocytes, osteoblasts and synovial cells that degrade type I, II and III collagen of the extracellular matrix and mediate in cartilage degeneration(60,61). In osteoarthrosis, MMP-1 is over-expressed in osteoarthrotic chondrocytes(62); MMP-3 is partly responsible for the degradation of non-collagen matrix proteins in rheumatoid arthritis and in osteoarthrosis(61), and an increased expression of MMP-3 and MMP-10 has been reported in joint cartilage and in the synovial membrane of osteoarthrotic patients. The rupture of collagen and aggrecan in cartilage is coordinated by MMP-9 and MMP-13. Severe rheumatoid arthritis is characterized by increased serum levels of MMP-9(63). In turn, col2 is degraded by MMP-1, 8, 13 and 14, and is partially degraded by gelatinases, MMP-2 and MMP-9 and MMP-3(64). A field for research and of possible future use is represented by the protease inhibitors, known as TIMP.
Tumour necrosis factor-α is an inflammatory factor that can activate certain cells to produce MMP(65), in relation to proteoglycan release from cartilage, contributing to the catabolic process of osteoarthrosis. The serum TNFα levels have been associated to the decrease in radiographic joint space and cartilage loss(66).
Cartilage oligomeric matrix protein (COMP), also known as thrombospondin-5, is a non-collagen protein that has been associated to cartilage degradation in osteoarthrosis(67). The serum concentration of COMP is sensitive to physical exercise(68). Liphardt et al.(69) reported a decrease in COMP, MMP-3 and MMP-9 after immobilization (bed rest) during 14 days, though the same was not observed for either TNFα or MMP-1.
Traumatisms are a triggering factor in the pathogenesis of knee osteoarthrosis; 50% of all patients with anterior cruciate ligament (ACL) rupture develop knee osteoarthrosis 10-15 years after surgery(70,71,72,73). This suggests that there are other less well known joint risk factors, in addition to joint instability, that affect the progression of osteoarthrosis.
Fluid ("wet") biomarkers
It has been seen that lumican and a 29 kDa metabolite of the latter enhance with the progression of osteoarthrosis(74,75). In turn, ADAMTS4, an aggrecanase synthesized by the osteoarthrotic synovial membrane, is associated to aggrecan degradation in the superficial zone of the joint cartilage(76), and is a marker of inflammation in the synovial fluid(77).
Metabolomics and proteomics
Metabolic fingerprinting has been studied in guinea pigs that develop spontaneous osteoarthrosis(78,79,80). Proteomic analysis of the osteoarthrotic cartilage secretome identifies molecules with functions in the disease process and allows global study of the secreted proteins and the identification of new biomarkers. A study in horses(81) documented a clear decrease in mean aggrecan G3 concentration with advancing age of the individual(82).
The proteomic profile of the synovial fluid in an osteoarthrotic and normal equine interphalangeal joint identified 754 proteins, of which 593 proved significant according to the Mascot scale. The identified proteins included those related to the matrix, inflammation, activation complements and proteases. A small group of 10 proteins was identified with a different expression in the osteoarthrotic synovial fluid that in future may prove to be candidate biomarkers of osteoarthrosis(81).
New generation sequencing may be used to identify small and unique genomic variations encrypted within each individual genome, and locate the active transcription genes in individual tissues(53). This allows detailed study of transcription and its correlation to the clinical symptoms, with a view to establishing a cause-effect relationship. This strategy could identify patterns associated with therapeutic interventions and final outcomes.
Gene therapy
Another possibility is offered by gene therapy. Although to date this field has not presented clear indications or conclusive results, one of its most promising perspectives corresponds to joint disease. Diarthroses are closed spaces characterized by tissues with a great cell differentiation capacity - adipose tissue cells, MSCs and synoviocytes - together with a rich synovial vascularization.
The aim of gene therapy is to stimulate the intrinsic cartilage repair activities from multipotent MSCs of the bone marrow(83). This can be done by applying the genic therapeutic vehicles directly within the lesion site(84), particularly vectors based on recombinant adeno-associated viruses (rAAV)(85), that support the transduction of these cells in a safe, effective and durable manner, without altering their chondrogenic potential(86). The chondrogenic candidates for effective gene therapy could be TGF-β, FGF-2, IGF-1 and SOX9(84,85,86,87,88).
Insulin-like growth factor 1 (IGF-1) is an anabolic factor for the treatment of osteoarthrosis. Gene therapy techniques were used in an equine model to produce IGF-1, together with a new generation of sequencers to establish a map of the biological response associated to the repair effects(89). Morscheid et al.(90) achieved over-expression of TGF with IGF-1 by means of rAAV that improved the anabolic, proliferative and chondrogenic activity of the cultured MSCs.
Mechanical regulation
Knowing and furthering understanding in biological mechanotransduction is one of the fields of greatest interest; it analyses the way in which mechanics influence the biology of hyaline cartilage, and how the effects of over-pressure and misalignment of the extremity can be avoided. Mechanotransduction modulates cell differentiation and genic expression; epigenetic differentiations of the genome itself may also occur(91).
We have underscored the biological and molecular aspects of cartilage repair. However, we also must take into account the mechanical influence upon cartilage and chondrocytes. The chondron unit responds in a way different from the isolated chondrocyte devoid of its pericellular matrix. The latter prepares the cell to respond to the mechanical demands. With advancing age, the chondrocytes are characterized by a lesser mechanical response, partly because the TGF-β signals switch from beneficial ALK-5/SMAD 2/3 to negative ALK-1/SMAD1/5/8(92).
It has been seen that the combination of compression and shear force demands upon the synovial fluid(93) improves cartilage morphology. These demands assist the MSCs during chondrogenesis, in the absence of growth factors, though no adequate response has been recorded with compression alone(94,95,96). However, chondrogenesis induced by MSCs through mechanical stimulation is not the same as when stimulation is made with other signals such as TGF-β, since mechanical chondrogenesis produces angiopoietin 2 (ANG2), osteoprotegerin (OPG) and nitric oxide (NO)(97).
Investigation of cartilage degeneration
Many experimental models have been used in the investigation of cartilage degeneration and osteoarthrosis. Most of the models make use of direct or traumatic mechanical overload to damage the cartilage in a short period of time. These studies in turn are carried out in the in vitro or in vivo setting. These are two completely different approaches, with different results; rather than being contradictory, however(98), they tend to complement each other.
In the case of in vitro studies, mechanical loading is the only experimental variable we can play with. Overloading cartilage explants in vitro allows us to establish its effects and the cartilage changes that occur over a short period of time. In turn, in vivo studies analyse the changes that occur over a few weeks to months or years after induction of the lesion. The biochemical changes are controlled in in vitro studies, but the main advantage of in vivo studies is that they afford a biomechanical and biological environment for studying joint degeneration in a whole joint, and in all its structures(98). In vitro studies seek to answer basic and elemental questions, while in vivo studies investigate responses under natural conditions and over a longer time interval.
Mechanical overload in vitro leads to immediate rupture of the most superficial layer of the cartilage(99,100) and to a decrease in cell viability(101). Necrosis and apoptotic cells increase following mechanical impact(102,103) and in all the impacted zone. In fact, the fissures produced by mechanical overload are always surrounded by dead cells(104,105,106), and following compression trauma, the apoptotic cells increase in number from the surface towards the more in-depth layers, in relation to the duration of compression. The release of prostaglandins (PGs) following overload occurs as a consequence of collagen network destructuring, which ruptures the PG chains and reduces their density(107). The production of glycosaminoglycans (GAGs) also decreases following high compression loads, with a decrease in cell viability(108,109) (Figure 3).
In vivo studies involving loading forces applied to cartilage seek to induce posttraumatic osteoarthrosis, using traumatism to analyse the progression of joint degeneration. Cartilage response to mechanical aggression can be studied directly (through impact or any other overloading protocol) or indirectly, altering joint kinematics, sectioning the anterior cruciate ligament, meniscectomy(113), osteotomies, or repeated overload during a prolonged period of time via muscle stimulation(114). These techniques produce changes in cartilage over time, with fissures, hypocellularity, loss of structural integrity, GAG elevation and subsequent reduction, and cartilage softening(115,116,117). With these models, the tibia is damaged to a greater degree than the femur, despite meniscal protection, and there is always less patellar degeneration, with a more pronounced effect in those zones that experience greater mechanical demand on walking. The production of fissures and GAG loss tend to worsen over time; timing of the study therefore must be adequately established, since greatly degenerated cartilage or scantly degenerated cartilage, in an animal model, contributes little to the study (Figure 4).
Conclusions
Many fields offering promising perspectives for cartilage repair remain open. All innovations should be based on new ideas, scientifically supported through experimental and clinical studies with adequate methodology, and published in reputed journals. This should serve to eliminate the existing "noise" generated by short-term impressions.
Growth factors are among the future lines of treatment, though we need to know both the doses and the mechanism of action in detail. There are a number of ways to proceed and different techniques can be used to deliver them to their targets and exert beneficial effects, with due control of the side effects in all cases.
Investigation should be based on anatomical, biological, cell and cell signal and biomechanical studies to analyse new implants and new surgeries. Attention should also be dedicated to diagnostic techniques in order to objectively assess follow-up and the final outcomes, based on imaging techniques and using specific biomarkers that are sensitive to any change in both the structure and composition of the joint cartilage.
Figuras
Figure 1. Anti-chondrogenic regulators in cartilage repair. The most important extra- and intracellular anti-chondrogenic regulators and their general mechanism of action. Modified from Lolli et al.(10).
Figure 2. Markers most widely used in inflammation and joint degeneration. Modified from Lotz et al.(57).
Figure 3. Schematic representation of long-term cartilage response according to the experimental overload methods used. Modified from Nickien et al.(98).
Información del artículo
Cita bibliográfica
Autores
Beatriz Bravo Molina
Facultad de Medicina. Universidad San Pablo-CEU. Campus de Montepríncipe. Boadilla del Monte, Madrid
Francisco Forriol Campos
Departamento de Ciencias Morfológicas y Cirugía. Universidad de Alcalá de Henares. Madrid.
Laboratorio de Ortopedia Experimental. Departamento de Cirugía Ortopédica y Traumatología. Clínica Universitaria. Facultad de Medicina. Universidad de Navarra. Pamplona
Laboratorio de Biomecánica. Clínica Universitaria de Navarra. Pamplona
Facultad de Medicina. Universidad San Pablo CEU. Campus de Montepríncipe. Boadilla del Monte. Madrid
Área de Investigación. Hospital FREMAP. Majadahonda. Madrid
Eduardo Álvarez Lozano
Hospital E. González. Universidad Autónoma de Nuevo León. Monterrey, México
Ethical responsibilities
Conflicts of interest. The authors declare that they have no conflicts of interest.
Financial support. This study has received no financial support.
Protection of people and animals. The authors declare that the procedures carried out abided with the ethical standards of the responsible human experimentation committee and in accordance with the World Medical Association and the Declaration of Helsinki.
Data confidentiality. The authors declare that the protocols of their centre referred to the publication of patient information have been followed.
Right to privacy and informed consent. The authors declare that no patient data appear in this article.
Referencias bibliográficas
-
1Awad ME, Hussein KA, Helwa I, Abdelsamid MF, Aguilar-Pérez A, Mohsen I, et al. Meta-Analysis and Evidence Base for the Efficacy of Autologous Bone Marrow Mesenchymal Stem Cells in Knee Cartilage Repair: Methodological Guidelines and Quality Assessment. Stem Cells Int. 2019 Apr 7;2019:3826054.
-
2Enea D, Cecconi S, Calcagno S, Busilacchi A, Manzotti S, Gigante A. One-step cartilage repair in the knee: collagen-covered microfracture and autologous bone marrow concentrate. A pilot study. Knee. 2015;22:30-5.
-
3Gobbi A, Scotti C, Karnatzikos G, Mudhigere A, Castro M, Peretti GM. One-step surgery with multipotent stem cells and Hyaluronan-based scaffold for the treatment of full-thickness chondral defects of the knee in patients older than 45 years. Knee Surg Sports Traumatol Arthrosc. 2017;25:2494-501.
-
4Haleem AM, Singergy AA, Sabry D, Atta HM, Rashed LA, Chu CR, et al. The clinical use of human culture-expanded autologous bone marrow mesenchymal stem cells transplanted on platelet-rich fibrin glue in the treatment of articular cartilage defects: a pilot study and preliminary results. Cartilage. 2010;1:253-61.
-
5Wong KL, Lee KB, Tai BC, Law P, Lee EH,Hui JH. Injectable cultured bone marrow-derived mesenchymal stem cells in varus knees with cartilage defects undergoing high tibial osteotomy: a prospective, randomized controlled clinical trial with 2 years' follow-up. Arthroscopy. 2013;29:2020-8.
-
6Buda R, Castagnini F, Cavallo M, Ramponi L, Vannini F, Giannini S. "One-step" bone marrow-derived cells transplantation and joint debridement for osteochondral lesions of the talus in ankle osteoarthritis: clinical and radiological outcomes at 36 months. Arch Orthop Trauma Surg. 2016;136:107-16.
-
7Nejadnik H, Hui JH, Feng Choong EP, Tai BC, Lee EH. Autologous bone marrow-derived mesenchymal stem cells versus autologous chondrocyte implantation: an observational cohort study. Am J Sports Med. 2010;38:1110-6.
-
8Stanish WD, McCormack R, Forriol F, Mohtadi N, Pelet S, Desnoyers J, et al. Novel scaffold-based BST-CarGel treatment results in superior cartilage repair compared with microfracture in a randomized controlled trial. J Bone Joint Surg Am. 2013;95:1640-50.
-
9Shive MS, Stanish WD, McCormack R, Forriol F, Mohtadi N, Pelet S, et al. BST-CarGel® Treatment Maintains Cartilage Repair Superiority over Microfracture at 5 Years in a Multicenter Randomized Controlled Trial. Cartilage. 2015;6:62-72.
-
10Lolli A, Colella F, De Bari C, van Osch GJVM. Targeting anti-chondrogenic factors for the stimulation of chondrogenesis: A new paradigm in cartilage repair. J Orthop Res. 2019;37:12-22.
-
11Scotti C, Tonnarelli B, Papadimitropoulos A, Scherberich A, Schaeren S, Schauerte A, et al. Recapitulation of endochondral bone formation using human adult mesenchymal stem cells as a paradigm for developmental engineering. Proc Natl Acad Sci U S A. 2010;107:7251-6.
-
12Pelttari K, Winter A, Steck E, Goetzke K, Hennig T, Ochs BG, et al. Premature induction of hypertrophy during in vitro chondrogenesis of human mesenchymal stem cells correlates with calcification and vascular invasion after ectopic transplantation in SCID mice. Arthritis Rheum. 2006;54:3254-66.
-
13Vangsness CT Jr, Farr J 2nd, Boyd J, Dellaero DT, Mills CR, LeRoux-Williams M. Adult human mesenchymal stem cells delivered via intra-articular injection to the knee following partial medial meniscectomy: a randomized, double-blind, controlled study. J Bone Joint Surg (Am). 2014;96-A:90-8.
-
14Forriol F. Growth factors in cartilage and meniscus repair. Injury. 2009;40(Suppl 3):S12-6.
-
15Vaquero J, Forriol F. Knee chondral injuries: clinical treatment strategies and experimental models. Injury. 2012;43:694-705.
-
16Curtin CM, Castaño IM, O'Brien FJ. Scaffold-Based microRNA Therapies in Regenerative Medicine and Cancer. Adv Healthc Mater. 2018;7(1).
-
17Chahla J, Dean CS, Moatshe G, Mitchell JJ, Cram TR, Yacuzzi C, et al. Meniscal Ramp Lesions: Anatomy, Incidence, Diagnosis, and Treatment. Orthop J Sports Med. 2016;4:2325967116657815.
-
18Esparza R, Gortazar AR, Forriol F. Cell study of the three areas of the meniscus: effect of growth factors in an experimental model in sheep. J Orthop Res. 2012;30:1647-51.
-
19Van der Kraan P, Matta C, Mobasheri A. Age-related alterations in signaling pathways in articular chondrocytes: implications for the pathogenesis and progression of osteoarthritis. A mini-review. Gerontology. 2017;63:29-35.
-
20Bakker AC, van de Loo FA, van Beuningen HM, Sime P, van Lent PL, van der Kraan PM, et al. Overexpression of active TGF-beta-1 in the murine knee joint: evidence for synovial-layer-dependent chondro-osteophyte formation. Osteoarthritis Cartilage. 2001;9:128-36.
-
21Van Beuningen HM, Glansbeek HL, van der Kraan PM, van den Berg WB. Differential effects of local application of BMP-2 or TGF-beta 1 on both articular cartilage composition and osteophyte formation. Osteoarthritis Cartilage. 1998;6:306-17.
-
22Izal I, Acosta CA, Ripalda P, Zaratiegui M, Ruiz J, Forriol F. IGF-1 gene therapy to protect articular cartilage in a rat model of joint damage. Arch Orthop Trauma Surg. 2008;128:239-47.
-
23Izal I, Ripalda P, Acosta CA, Forriol F. In vitro healing of avascular meniscal injuries with fresh and frozen plugs treated with TGF-beta1 and IGF-1 in sheep. Int J Clin Exp Pathol. 2008;1:426-34.
-
24Enomoto-Iwamoto M, Nakamura T, Aikawa T, Higuchi Y, Yuasa T, Yamaguchi A, et al. Hedgehog proteins stimulate chondrogenic cell differentiation and cartilage formation. J Bone Miner Res. 2000;15:1659-68.
-
25Weiss S, Hennig T, Bock R, Steck E, Richter W. Impact of growth factors and PTHrP on early and late chondrogenic differentiation of human mesenchymal stem cells. J Cell Physiol. 2010;223:84-93.
-
26Hellingman CA, Koevoet W, Kops N, Farrell E, Jahr H, Liu W, et al. Fibroblast growth factor receptors in in vitro and in vivo chondrogenesis: relating tissue engineering using adult mesenchymal stem cells to embryonic development. Tissue Eng Part A. 2010;16:545-56.
-
27Miljkovic ND, Cooper GM, Marra KG. Chondrogenesis, bone morphogenetic protein-4 and mesenchymal stem cells. Osteoarthritis Cartilage. 2008;16:1121-30.
-
28Green JD, Tollemar V, Dougherty M, Yan Z, Yin L, Ye J, et al. Multifaceted signaling regulators of chondrogenesis: Implications in cartilage regeneration and tissue engineering. Genes Dis. 2015;2:307-27.
-
29Watanabe N, Tezuka Y, Matsuno K, Miyatani S, Morimura N, Yasuda M, et al. Suppression of differentiation and proliferation of early chondrogenic cells by Notch. J Bone Miner Metab. 2003;21:344-52.
-
30Goldring SR, Goldring MB. The role of cytokines in cartilage matrix degeneration in osteoarthritis. Clin Orthop Relat Res. 2004;427(Suppl):S27-36.
-
31Schmidt TA, Gastelum NS, Nguyen QT, Schumacher BL, Sah RL. Boundary lubrication of articular cartilage: role of synovial fluid constituents. Arthritis Rheum. 2007;56:882-91.
-
32Bonnevie ED, Galesso D, Secchieri C, Bonassar LJ. Degradation alters the lubrication of articular cartilage by high viscosity, hyaluronic acid-based lubricants. J Orthop Res. 2018;36:1456-64.
-
33Greene GW, Banquy X, Lee DW, Lowrey DD, Yu J, Israelachvili JN. Adaptive mechanically controlled lubrication mechanism found in articular joints. Proc Natl Acad Sci U S A. 2011;108:5255-9.
-
34Arner EC, Hughes CE, Decicco CP, Caterson B, Tortorella MD. Cytokine-induced cartilage proteoglycan degradation is mediated by aggrecanase. Osteoarthritis Cartilage. 1998;6:214-28.
-
35Sandy JD, Thompson V, Verscharen C, Gamett D. Chondrocyte-mediated catabolism of aggrecan: evidence for a glycosylphosphatidylinositol-linked protein in the aggrecanase response to interleukin-1 or retinoic acid. Arch Biochem Biophys. 1999;367:258-64.
-
36Baici A, Lang A. Effect of interleukin-1 beta on the production of cathepsin B by rabbit articular chondrocytes. FEBS Lett. 1990;277:93-6.
-
37Lee Y, Choi J, Hwang NS. Regulation of lubricin for functional cartilage tissue regeneration: a review. Biomater Res. 2018 Mar 16;22:9.
-
38Elsaid KA, Jay GD, Chichester CO. Reduced expression and proteolytic susceptibility of lubricin/superficial zone protein may explain early elevation in the coefficient of friction in the joints of rats with antigen-induced arthritis. Arthritis Rheum. 2007;56:108-16.
-
39Gleghorn JP, Jones AR, Flannery CR, Bonassar LJ. Alteration of articular cartilage frictional properties by transforming growth factor beta, interleukin-1beta, and oncostatin M. Arthritis Rheum. 2009;60:440-9.
-
40Larson KM, Zhang L, Elsaid KA, Schmidt TA, Fleming BC, Badger GJ, et al. Reduction of friction by recombinant human proteoglycan 4 in IL-1α stimulated bovine cartilage explants. J Orthop Res. 2017;35:580-9.
-
41Zhang Y, Pizzute T, Pei M. Anti-inflammatory strategies in cartilage repair. Tissue Eng Part B Rev. 2014;20:655-68.
-
42Fahy N, Farrell E, Ritter T, Ryan AE, Murphy JM. Immune modulation to improve tissue engineering outcomes for cartilage repair in the osteoarthritic joint. Tissue Eng Part B Rev. 2015;21:55-66.
-
43Zhong W, Zhang W, Wang S, Qin J. Regulation of fibrochondrogenesis of mesenchymal stem cells in an integrated microfluidic platform embedded with biomimetic nanofibrous scaffolds. PLoS One. 2013;8:e61283.
-
44Karystinou A, Roelofs AJ, Neve A, Cantatore FP, Wackerhage H, De Bari C. Yes-associated protein (YAP) is a negative regulator of chondrogenesis in mesenchymal stem cells. Arthritis Res Ther. 2015;17:147.
-
45Gabler J, Ruetze M, Kynast KL, Grossner T, Diederichs S, Richter W. Stage-Specific miRs in Chondrocyte Maturation: Differentiation-Dependent and Hypertrophy-Related miR Clusters and the miR-181 Family. Tissue Eng Part A. 2015;21:2840-51.
-
46Diederichs S, Gabler J, Autenrieth J, Kynast KL, Merle C, Walles H, et al. Differential Regulation of SOX9 Protein During Chondrogenesis of Induced Pluripotent Stem Cells Versus Mesenchymal Stromal Cells: a Shortcoming for Cartilage Formation. Stem Cells Dev. 2016;25:598-609.
-
47Chang T, Xie J, Li H, Li D, Liu P, Hu Y. MicroRNA-30a promotes extracellular matrix degradation in articular cartilage via downregulation of Sox9. Cell Prolif. 2016;49:207-18.
-
48Mahajan TD, Mikuls TR. Recent advances in the treatment of rheumatoid arthritis. Curr Opin Rheumatol. 2018;30:231-7.
-
49De Aguiar RB, Parise CB, Souza CR, Braggion C, Quintilio W, Moro AM, et al. Blocking FGF2 with a new specific monoclonal antibody impairs angiogenesis and experimental metastatic melanoma, suggesting a potential role in adjuvant settings. Cancer Lett. 2016;371:151-60.
-
50Yoshizuka M, Nakasa T, Kawanishi Y, Hachisuka S, Furuta T, Miyaki S, et al. Inhibition of microRNA-222 expression accelerates bone healing with enhancement of osteogenesis, chondrogenesis, and angiogenesis in a rat refractory fracture model. J Orthop Sci. 2016;21:852-8.
-
51Lin J, Teo S, Lam DH, Jeyaseelan K, Wang S. MicroRNA-10b pleiotropically regulates invasion, angiogenicity and apoptosis of tumor cells resembling mesenchymal subtype of glioblastoma multiforme. Cell Death Dis. 2012;3:e398.
-
52Connelly CM, Deiters A. Control of oncogenic miRNA function by light-activated miRNA antagomirs. Methods Mol Biol. 2014;1165:99-114.
-
53McIlwraith CW, Kawcak CE, Frisbie DD, Little CB, Clegg PD, Peffers MJ, et al. Biomarkers for equine joint injury and osteoarthritis. J Orthop Res. 2018;36:823-31.
-
54Kraus VB, Burnett B, Coindreau J, Cottrell S, Eyre D, Gendreau M, et al.; OARSI FDA Osteoarthritis Biomarkers Working Group. Application of biomarkers in the development of drugs intended for the treatment of osteoarthritis. Osteoarthritis Cartilage. 2011;19:515-42.
-
55Kraus VB, Blanco FJ, Englund M, Henrotin Y, Lohmander LS, Losina E, et al. OARSI Clinical Trials Recommendations: Soluble biomarker assessments in clinical trials in osteoarthritis. Osteoarthritis Cartilage. 2015;23:686-97.
-
56Karsdal MA, Byrjalsen I, Alexandersen P, Bihlet A, Andersen JR, Riis BJ, et al.; CSMC021C2301/2 investigators. Treatment of symptomatic knee osteoarthritis with oral salmon calcitonin: results from two phase 3 trials. Osteoarthritis Cartilage. 2015;23:532-43.
-
57Lotz M, Martel-Pelletier J, Christiansen C, Brandi ML, Bruyère O, Chapurlat R, et al. Republished: Value of biomarkers in osteoarthritis: current status and perspectives. Postgrad Med J. 2014;90:171-8.
-
58Yong VW, Agrawal SM, Stirling DP. Targeting MMPs in acute and chronic neurological conditions. Neurotherapeutics. 2007;4:580-9.
-
59Morrison CJ, Butler GS, Rodríguez D, Overall CM. Matrix metalloproteinase proteomics: substrates, targets, and therapy. Curr Opin Cell Biol. 2009;21:645-53.
-
60Valdes AM, Spector TD. Genetic epidemiology of hip and knee osteoarthritis. Nat Rev Rheumatol. 2011;7:23-32.
-
61Burrage PS, Mix KS, Brinckerhoff CE. Matrix metalloproteinases: role in arthritis. Front Biosci. 2006;11:529-43.
-
62Bravo B, Argüello JM, Gortazar AR, Forriol F, Vaquero J. Modulation of Gene Expression in Infrapatellar Fat Pad-Derived Mesenchymal Stem Cells in Osteoarthritis. Cartilage. 2018;9:55-62.
-
63Gruber BL, Sorbi D, French DL, Marchese MJ, Nuovo GJ, Kew RR, et al. Markedly elevated serum MMP-9 (gelatinase B) levels in rheumatoid arthritis: a potentially useful laboratory marker. Clin Immunol Immunopathol. 1996;78:161-71.
-
64De Ceuninck F, Sabatini M, Pastoureau P. Recent progress toward biomarker identification in osteoarthritis. Drug Discov Today. 2011;16:443-9.
-
65Cawston TE, Wilson AJ. Understanding the role of tissue degrading enzymes and their inhibitors in development and disease. Best Pract Res Clin Rheumatol. 2006;20:983-1002.
-
66Daghestani HN, Kraus VB. Inflammatory biomarkers in osteoarthritis. Osteoarthritis Cartilage. 2015;23:1890-6.
-
67Saxne T, Heinegård D. Synovial fluid analysis of two groups of proteoglycan epitopes distinguishes early and late cartilage lesions. Arthritis Rheum. 1992;35:385-90.
-
68Niehoff A, Kersting UG, Helling S, Dargel J, Maurer J, Thevis M, et al. Different mechanical loading protocols influence serum cartilage oligomeric matrix protein levels in young healthy humans. Eur J Appl Physiol. 2010;110:651-7.
-
69Liphardt AM, Mündermann A, Andriacchi TP, Achtzehn S, Heer M, Mester J. Sensitivity of serum concentration of cartilage biomarkers to 21-days of bed rest. J Orthop Res. 2018;36:1465-71.
-
70Noé B, Poole AR, Mort JS, Richard H, Beauchamp G, Laverty S. C2K77 ELISA detects cleavage of type II collagen by cathepsin K in equine articular cartilage. Osteoarthritis Cartilage. 2017;25:2119-26.
-
71Frobell RB, Roos EM, Roos HP, Ranstam J, Lohmander LS. A randomized trial of treatment for acute anterior cruciate ligament tears. N Engl J Med. 2010;363:331-42.
-
72Delincé P, Ghafil D. Anterior cruciate ligament tears: conservative or surgical treatment? A critical review of the literature. Knee Surg Sports Traumatol Arthrosc. 2012;20:48-61.
-
73Claes S, Hermie L, Verdonk R, Bellemans J, Verdonk P. Is osteoarthritis an inevitable consequence of anterior cruciate ligament reconstruction? A meta-analysis. Knee Surg Sports Traumatol Arthrosc. 2013;21:1967-76.
-
74Frisbie DD, Mc Ilwraith CW, Arthur RM, Blea J, Baker VA, Billinghurst RC. Serum biomarker levels for musculoskeletal disease in two- and three-year-old racing Thoroughbred horses: a prospective study of 130 horses. Equine Vet J. 2010;42:643-51.
-
75Melrose J, Fuller ES, Roughley PJ, Smith MM, Kerr B, Hughes CE, et al. Fragmentation of decorin, biglycan, lumican and keratocan is elevated in degenerate human meniscus, knee and hip articular cartilages compared with age-matched macroscopically normal and control tissues. Arthritis Res Ther. 2008;10:R79.
-
76Young AA, Smith MM, Smith SM, Cake MA, Ghosh P, Read RA, et al. Regional assessment of articular cartilage gene expression and small proteoglycan metabolism in an animal model of osteoarthritis. Arthritis Res Ther. 2005;7:R852-61.
-
77Wainwright SD, Bondeson J, Caterson B, Hughes CE. ADAMTS-4_v1 is a splice variant of ADAMTS-4 that is expressed as a protein in human synovium and cleaves aggrecan at the interglobular domain. Arthritis Rheum. 2013;65:2866-75.
-
78Reo NV. NMR-based metabolomics. Drug Chem Toxicol. 2002;25:375-82.
-
79Huebner JL, Hanes MA, Beekman B, TeKoppele JM, Kraus VB. A comparative analysis of bone and cartilage metabolism in two strains of guinea-pig with varying degrees of naturally occurring osteoarthritis. Osteoarthritis Cartilage. 2002;10:758-67.
-
80Bravo B, Guisasola MC, Vaquero J, Tirado I, Gortazar AR, Forriol F. Gene expression, protein profiling, and chemotactic activity of infrapatellar fat pad mesenchymal stem cells in pathologies of the knee joint. J Cell Physiol. 2019;234:18917-27.
-
81Peffers MJ, McDermott B, Clegg PD, Riggs CM. Comprehensive protein profiling of synovial fluid in osteoarthritis following protein equalization. Osteoarthritis Cartilage. 2015;23:1204-13.
-
82Beynon RJ, Doherty MK, Pratt JM, Gaskell SJ. Multiplexed absolute quantification in proteomics using artificial QCAT proteins of concatenated signature peptides. Nat Methods. 2005;2:587-9.
-
83Orth P, Rey-Rico A, Venkatesan JK, Madry H, Cucchiarini M. Current perspectives in stem cell research for knee cartilage repair. Stem Cells Cloning. 2014;7:1-17.
-
84Frisch J, Venkatesan JK, Rey-Rico A, Madry H, Cucchiarini M. Current progress in stem cell-based gene therapy for articular cartilage repair. Curr Stem Cell Res Ther. 2015;10:121-31.
-
85Cucchiarini M. Human gene therapy: novel approaches to improve the current gene delivery systems. Discov Med. 2016;21:495-506.
-
86Tao K, Frisch J, Rey-Rico A, Venkatesan JK, Schmitt G, Madry H, et al. Co-overexpression of TGF-β and SOX9 via rAAV gene transfer modulates the metabolic and chondrogenic ativities of human bone marrow-derived mesenchymal stem cells. Stem Cell Res Ther. 2016;7:20-31.
-
87Pagnotto MR, Wang Z, Karpie JC, Ferretti M, Xiao X, Chu CR. Adeno-associated viral gene transfer of transforming growth factor-beta1 to human mesenchymal stem cells improves cartilage repair. Gene Ther. 2007;14:804-13.
-
88Venkatesan JK, Ekici M, Madry H, Schmitt G, Kohn D, et al. SOX9 gene transfer via safe, stable, replication-defective recombinant adeno-associated virus vectors as a novel, powerful tool to enhance the chondrogenic potential of human mesenchymal stem cells. Stem Cell Res Ther. 2012;3:22-36.
-
89O'Hara S, Wang K, Slayden RA, Schenkel AR, Huber G, O'Hern CS, et al. Iterative feature removal yields highly discriminative pathways. BMC Genomics. 2013;14:832.
-
90Morscheid S, Rey-Rico A, Schmitt G, Madry H, Cucchiarini M, Venkatesan JK. Therapeutic Effects of rAAV-Mediated Concomittant Gene Transfer and Overexpression of TGF-β and IGF-I on the Chondrogenesis of Human Bone-Marrow-Derived Mesenchymal Stem Cells. Int J Mol Sci. 2019 May 27;20(10):2591.
-
91Guo XE, Hung CT, Sandell LJ, Silva MJ. Musculoskeletal mechanobiology: a new era for mechano medicine. J Orthop Res. 2018;35:531-2.
-
92Madej W, van Caam A, Davidson EN, Hannink G, Buma P, van der Kraan PM. Aging is associated with reduction of mechanically-induced activation of Smad2/3P signaling in articular cartilage. Osteoarthritis Cartilage. 2016;24:146-57.
-
93Albro MB, Cigan AD, Nims RJ, Yeroushalmi KJ, Oungoulian SR, Hung CT, et al. Shearing of synovial fluid activates latent TGF-β. Osteoarthritis Cartilage. 2012;20:1374-82.
-
94Li Z, Kupcsik L, Yao SJ, Alini M, Stoddart MJ. Mechanical load modulates chondrogenesis of human mesenchymal stem cells through the TGF-beta pathway. J Cell Mol Med. 2010;14:1338-46.
-
95Schätti O, Grad S, Goldhahn J, Salzmann G, Li Z, Alini M, et al. A combination of shear and dynamic compression leads to mechanically induced chondrogenesis of human mesenchymal stem cells. Eur Cell Mater. 2011;22:214-25.
-
96Glatt V, Evans CH, Stoddart MJ. Regenerative rehabilitation: the role of mechanotransduction in orthopaedic regenerative medicine. J Orthop Res. 2019;37:1263-9.
-
97Gardner OF, Fahy N, Alini M, Stoddart MJ. Differences in human mesenchymal stem cell secretomes during chondrogenic induction. Eur Cell Mater. 2016;31:221-35.
-
98Nickien M, Heuijerjans A, Ito K, van Donkelaar CC. Comparison between in vitro and in vivo cartilage overloading studies based on a systematic literature review. J Orthop Res. 2018 Apr 12;36(8):2076-86.
-
99Leucht F, Dürselen L, Hogrefe C, Joos H, Reichel H, Schmitt H, et al. Development of a new biomechanically defined single impact rabbit cartilage trauma model for in vivo-studies. J Invest Surg. 2012;25:235-41.
-
100Malekipour F, Whitton C, Oetomo D, Lee PV. Shock absorbing ability of articular cartilage and subchondral bone under impact compression. J Mech Behav Biomed Mater. 2013;26:127-35.
-
101Jang KW, Buckwalter JA, Martin JA. Inhibition of cell-matrix adhesions prevents cartilage chondrocyte death following impact injury. J Orthop Res. 2014;32:448-54.
-
102Chen CT, Burton-Wurster N, Borden C, Hueffer K, Bloom SE, Lust G. Chondrocyte necrosis and apoptosis in impact damaged articular cartilage. J Orthop Res. 2001;19:703-11.
-
103Patwari P, Gaschen V, James IE, Berger E, Blake SM, Lark MW, et al. Ultrastructural quantification of cell death after injurious compression of bovine calf articular cartilage. Osteoarthritis Cartilage. 2004;12:245-52.
-
104Alexander PG, Song Y, Taboas JM, Chen FH, Melvin GM, Manner PA, et al. Development of a Spring-Loaded Impact Device to Deliver Injurious Mechanical Impacts to the Articular Cartilage Surface. Cartilage. 2013;4:52-62.
-
105Stolberg-Stolberg JA, Furman BD, Garrigues NW, Lee J, Pisetsky DS, Stearns NA, et al. Effects of cartilage impact with and without fracture on chondrocyte viability and the release of inflammatory markers. J Orthop Res. 2013;31:1283-92.
-
106Backus JD, Furman BD, Swimmer T, Kent CL, McNulty AL, Defrate LE, et al. Cartilage viability and catabolism in the intact porcine knee following transarticular impact loading with and without articular fracture. J Orthop Res. 2011;29:501-10.
-
107Rolauffs B, Muehleman C, Li J, Kurz B, Kuettner KE, Frank E, et al. Vulnerability of the superficial zone of immature articular cartilage to compressive injury. Arthritis Rheum. 2010;62:3016-27.
-
108Piscoya JL, Fermor B, Kraus VB, Stabler TV, Guilak F. The influence of mechanical compression on the induction of osteoarthritis-related biomarkers in articular cartilage explants. Osteoarthritis Cartilage. 2005;13:1092-9.
-
109Clements KM, Bee ZC, Crossingham GV, Adams MA, Sharif M. How severe must repetitive loading be to kill chondrocytes in articular cartilage? Osteoarthritis Cartilage. 2001;9:499-507.
-
110Borrelli J Jr, Silva MJ, Zaegel MA, Franz C, Sandell LJ. Single high-energy impact load causes posttraumatic OA in young rabbits via a decrease in cellular metabolism. J Orthop Res. 2009;27:347-52.
-
111Thibault M, Poole AR, Buschmann MD. Cyclic compression of cartilage/bone explants in vitro leads to physical weakening, mechanical breakdown of collagen and release of matrix fragments. J Orthop Res. 2002;20:1265-73.
-
112Cho H, Pinkhassik E, David V, Stuart JM, Hasty KA. Detection of early cartilage damage using targeted nanosomes in a post-traumatic osteoarthritis mouse model. Nanomedicine. 2015 May;11(4):939-46.
-
113Arunakul M, Tochigi Y, Goetz JE, Diestelmeier BW, Heiner AD, Rudert J, et al. Replication of chronic abnormal cartilage loading by medial meniscus destabilization for modeling osteoarthritis in the rabbit knee in vivo. J Orthop Res. 2013;31:1555-60.
-
114Horisberger M, Fortuna R, Valderrabano V, Herzog W. Long-term repetitive mechanical loading of the knee joint by in vivo muscle stimulation accelerates cartilage degeneration and increases chondrocyte death in a rabbit model. Clin Biomech (Bristol, Avon). 2013;28:536-43.
-
115Du G, Zhan H, Ding D, Wang S, Wei X, Wei F, et al. Abnormal Mechanical Loading Induces Cartilage Degeneration by Accelerating Meniscus Hypertrophy and Mineralization After ACL Injuries In Vivo. Am J Sports Med. 2016;44:652-63.
-
116Desando G, Giavaresi G, Cavallo C, Bartolotti I, Sartoni F, Nicoli Aldini N, et al. Autologous Bone Marrow Concentrate in a Sheep Model of Osteoarthritis: New Perspectives for Cartilage and Meniscus Repair. Tissue Eng Part C Methods. 2016;22:608-19.
-
117Burger C, Mueller M, Wlodarczyk P, Goost H, Tolba RH, Rangger C, et al. The sheep as a knee osteoarthritis model: early cartilage changes after meniscus injury and repair. Lab Anim. 2007;41:420-31.
Descargar artículo:

Licencia:
Este contenido es de acceso abierto (Open-Access) y se ha distribuido bajo los términos de la licencia Creative Commons CC BY-NC-ND (Reconocimiento-NoComercial-SinObraDerivada 4.0 Internacional) que permite usar, distribuir y reproducir en cualquier medio siempre que se citen a los autores y no se utilice para fines comerciales ni para hacer obras derivadas.
Comparte este contenido
En esta edición
- Update on the treatment of focal cartilage lesions of the knee
- Comparison of the intraarticular injection of platelet rich plasma (PRGF®) and hyaluronic acid (Hyalone®) in the treatment of chondral lesions: a randomized, prospective clinical study
- Microfractures or bone marrow stimulation (BMS): evolution of the technique
- Fresh osteochondral graft. Indications, surgical technique and scientific evidence
- Autologous matrix-induced chondrogenesis (AMIC)
- Instant CEMTRO Cell (ICC), high density autologous chondrocytes implantation
- Regeneration of joint cartilage: perspectives and future
- Autologous chondrocyte implant surgery of the knee
- Full thickness chondral ulcers of the patella and femoral condyle of the knee
Más en PUBMED
Más en Google Scholar
Más en ORCID
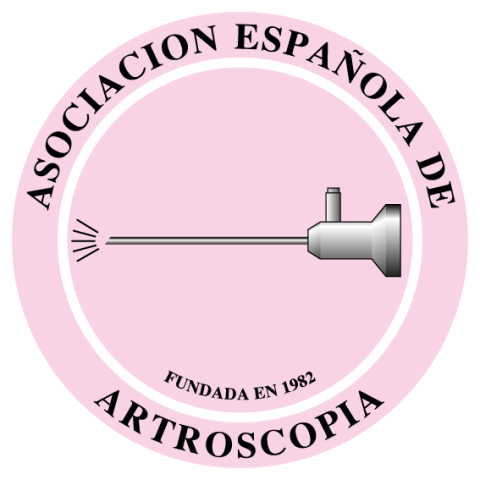

Revista Española de Artroscopia y Cirugía Articular está distribuida bajo una licencia de Creative Commons Reconocimiento-NoComercial-SinObraDerivada 4.0 Internacional.